CAZypedia needs your help!
We have many unassigned pages in need of Authors and Responsible Curators. See a page that's out-of-date and just needs a touch-up? - You are also welcome to become a CAZypedian. Here's how.
Scientists at all career stages, including students, are welcome to contribute.
Learn more about CAZypedia's misson here and in this article.
Totally new to the CAZy classification? Read this first.
Difference between revisions of "Carbohydrate-binding modules"
Line 59: | Line 59: | ||
''CBM Promiscuity'' | ''CBM Promiscuity'' | ||
− | Because of the diversity of carbohydrate structures and motifs found in plant and mammalian glycans, some CBMs have become adapted to recognize more than one type of monosaccharide or glycosidic bond linkage within the binding pocket, a feature called CBM promiscuity. For example a family CBM32 from ''Clostridium perfringens'' NagH binds N-acetyl-glucosamine in the primary subsite but can accommodate N-acetyl-galactosamine or mannose in the secondary site <cite>Ficko20092</cite>. There are several examples of ligand promiscuity within family [[CBM32]]. In plant cell wall recognizing CBMs, they are often able to accommodate both cellulose and hemicelluloses. For example, several family CBM6 members interact with cellulose, xylose or laminarin <cite>Boraston20032 Lammerts2005</cite>. A family CBM41 can accommodate both alpha-1,4- and alpha-1,6-linked glucose found in amylopectin (from starch/glycogen) <cite>Lammerts2007</cite>. The flexibility | + | Because of the diversity of carbohydrate structures and motifs found in plant and mammalian glycans, some CBMs have become adapted to recognize more than one type of monosaccharide or glycosidic bond linkage within the binding pocket, a feature called CBM promiscuity. For example a family CBM32 from ''Clostridium perfringens'' NagH binds N-acetyl-glucosamine in the primary subsite but can accommodate N-acetyl-galactosamine or mannose in the secondary site <cite>Ficko20092</cite>. There are several examples of ligand promiscuity within family [[CBM32]]. In plant cell wall recognizing CBMs, they are often able to accommodate both cellulose and hemicelluloses. For example, several family CBM6 members interact with cellulose, xylose or laminarin <cite>Boraston20032 Lammerts2005</cite>. A family CBM41 can accommodate both alpha-1,4- and alpha-1,6-linked glucose found in amylopectin (from starch/glycogen) <cite>Lammerts2007</cite>. The flexibility in carbohydrate recognition by CBMs contributes to the [[#Functional Roles of CBMs|targeting]] efficiency of carbohydrate-active enzymes in environments where there is diverse range of polysaccharides present (such as the plant cell wall or mammalian tissues). |
=== CBMs and Multivalency === | === CBMs and Multivalency === |
Revision as of 02:32, 15 July 2013
This page is currently under construction. This means that the Responsible Curator has deemed that the page's content is not quite up to CAZypedia's standards for full public consumption. All information should be considered to be under revision and may be subject to major changes.
- Author: ^^^Alicia Lammerts van Bueren^^^
- Responsible Curator: ^^^Al Boraston^^^ and ^^^Spencer Williams^^^
This page is under construction. In the meantime, please see these references for an essential introduction to the CAZy classification system: [1, 2]. CBMs, in particular, have been extensively reviewed[3, 4, 5, 6].
Overview
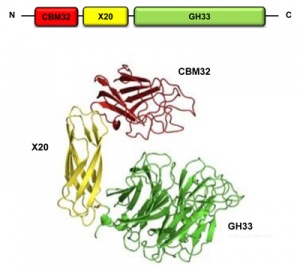
Carbohydrate-binding modules (CBMs) are defined as a stretch of amino acid sequence within a larger encoded protein sequence and fold into a discreet and independent module, forming part of a larger multi-modular protein (Figure 1). The role of a CBM is to bind to carbohydrate ligand and direct the catalytic machinery onto its substrate, thus enhancing the catalytic efficiency of the multimodular carbohydrate-active enzyme. CBMs are themselves devoid of any catalytic activity. CBMs are most commonly associated with Glycoside Hydrolases but have also been identified in Polysaccharide Lyases, polysaccharide oxidases, Glycosyltransferases and plant cell wall-binding expansins [8].
CBMs themselves do not undergo any conformational changes when binding ligand. Rather, the topography of the carbohydrate-binding site is preformed to be complementary to the shape of the target ligand (see Types). This is achieved by the presence of amino acid side chains and loops within the CBM binding pocket. However multimodular enzymes as a whole may be quite flexible and experience complete conformational changes when binding substrate. Flexible loop regions between adjacent modules can allow for shifts in the orientation and direction of the catalytic module with respect to the CBM on the target substrate [9].
History of CBMs
CBMs were initially characterized as cellulose binding domains (CBDs) in cellobiohydrolases CBHI and CBHII from Trichoderma reesei [10, 11] and cellulases CenA and CexA from Cellulomonas fimi [12]. Limited proteolysis experiments of these enzymes yielded truncated enzyme products that showed a reduced or complete loss in their ability to hydrolyze cellulose substrates. The reduction in enzymatic activity was attributed to the loss of ~100 amino acid C-terminal domains which prevented the adsorbption of the enzymes onto cellulose substrate. Thus it was proposed that these independent "domains" are critical for targeting the enzymes onto its substrate and enhancing their hydrolytic activity.
CBDs were previously categorized into 13 Types based on amino acid sequence similarities [13]. This classification system became complicated when similar functional domains from non-cellulolytic carbohydrate-active enzymes were discovered that did not bind cellulose but met all of the criteria of a CBD. The term carbohydrate-binding module was proposed to solve this problem to be inclusive of all ancillary modules with non-catalytic carbohydrate-binding function (for a review see [3]).
Classification
Sequence Based Classification
Carbohydrate-binding modules are currently classified into 67 families based on amino acid sequence similarities (May 2013), which are available through the Carbohydrate Active enZyme database. Sequence-based relationships often cluster together modules with similar structural folds and carbohydrate-binding function. While this is true for most CBM families, there are several families that exhibit diversity in the carbohydrate ligands they target (examples include CBM6, CBM32).
Fold
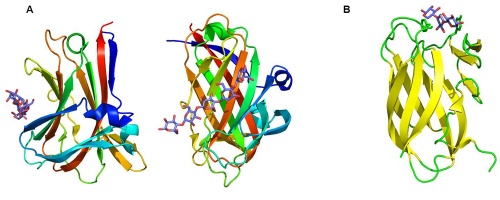
Structural information for 54 of the 67 CBM families is known. CBMs fall into one of 7 fold families [3]. The most common fold exhibited by CBMs is the beta-sandwich fold which is comprised of two overlapping beta-sheets consisting of three to six antiparallel beta strands (Figure 2). The ligand binding site is located primarily on the same face of a beta-sheet (Figure 2A), but may also be positioned on the edge of the beta-sheet within the joining loop region (Figure 2B). There are examples of CBMs in the beta-sandwich fold family exhibiting dual binding sites such as CBM6 [16] and dual starch-binding sites in CBM20 [17]. Other fold families include the beta-trefoil fold, cysteine knot, OB fold, the hevein and hevein-like and unique folds [3]. CBMs of the beta-trefoil fold family (CBM13, CBM42) present multivalent sugar-binding sites, as demonstrated for their interaction with xylan and arabinoxylan respectively [18].
Types
CBMs are classified into three main Types defined by the shape and degree of polymerization of their target ligand (Figure 3A). The architecture of the binding site determines what region within a polysaccharide the enzyme will target. A recent review on CBM plant cell wall recognition [20] has modified the classification of CBM Types to be as follows:
- Type A: bind to crystalline surfaces of cellulose and chitin (example families CBM1, CBM2, CBM3, CBM5, CBM10). Their binding sites are planar and rich in aromatic amino acid residues creating a flat platform to bind to the flat polycrystalline chitin/cellulose surface (Figure 3B). Type A CBMs are unique and differ significantly from Type B or C.
- Type B: bind internal glycan chains (endo-type). The most abundant CBM type. Type B binding sites appear as extended grooves or clefts comprised of binding subsites to accommodate longer sugar chains with four or more monosaccharide units (see Figure 2A for an example).
- Type C: bind termini of glycans (reducing/non-reducing ends, exo-type). Type C binding sites are short pockets for recognizing short sugar ligands containing one to three monosaccharide units (example families CBM9, CBM13, CBM32, CBM47). Families containing Type C CBMs often include lectins as members.
Properties of CBM Carbohydrate Binding Interactions
Functional Roles of CBMs
CBMs carry out four main functional roles:
- Targeting Effect: CBMs target the enzyme to distinct regions within a larger macromolecular polysaccharide substrate (reducing end, non-reducing end, internal polysaccharide chains), depending on the architecture of its binding site (see Types).
- Proximity Effect: CBMs increase the concentration of enzyme in close proximity to its polysaccharide substrate. This leads to more rapid and efficient substrate degradation.
An excellent example demonstrating targeting and proximity effects of plant cell wall specific CBMs is available [21].
- Disruptive Effect: Some CBMs have been shown to disrupt the surface of tightly packed polysaccharides, such as cellulose fibres and starch granules, causing the substrate to loosen and become more exposed to the catalytic module for more efficient degradation. Disruptive roles have been described for cellulose binding CBM2a [22] and CBM44 [23]. Dual starch-binding domains of family CBM20 from Aspergillus niger glucoamylase have been shown to disrupt the surface of starch [24] while dual-associated CBM41 modules may have a disruptive role in degrading glycogen granules [25]. CBM33 was thought to have a disruptive effect on chitin, however these have now been reclassified as Copper-dependent lytic polysaccharide monooxygenases [26] and are reclassified as Auxillary Activity Family 9.
- Adhesion: CBMs have been shown to adhere enzymes onto the surface of bacterial cell wall components while exhibiting catalytic activity on an external neighboring carbohydrate substrate. For example, CBM35 modules have been shown to interact with the surface glucuronic acid containing sugars in the cell wall of Amycolatopsis orientalis while the catalytic module is active on external chitosan likely originating from the cell wall of competing soil fungal species [27].
Driving Forces of CBM/Carbohydrate Interactions
There are two key features that drive CBM/carbohydrate interactions. Extensive hydrogen bonding occurs between the hydroxyl groups of carbohydrate ligands and polar amino acid residues within the binding site. Additional water-mediated hydrogen bonding networks between these groups can also be found in the binding site. By far the most important characteristic driving force mediating protein-carbohydrate interactions is the position and orientation of aromatic amino acid residues (Try, Tyr and sometimes Phe) within the binding site. These essential planar residues form hydrophobic stacking interactions with the planar face of sugar rings. Weak intermolecular electrostatic interactions occur between C-H and pi electrons in the planar ring systems and contribute 1.5 - 2.5 kcal/mol energy to the binding reaction [28].
CBMs may also use coordinated metal ions within the binding site to directly interact with their target ligand. For example, families CBM36 [29] and CBM60 [30] exhibit calcium-dependent binding to xylooligosaccharides.
CBM-carbohydrate interactions in general are quite weak (Ka affinities in mM-1 to uM-1 range) making the interaction easily reversible. This feature allows for "recycling" of the appended enzyme to bind to a new region on the substrate once catalysis has been completed at a given site.
CBM Promiscuity
Because of the diversity of carbohydrate structures and motifs found in plant and mammalian glycans, some CBMs have become adapted to recognize more than one type of monosaccharide or glycosidic bond linkage within the binding pocket, a feature called CBM promiscuity. For example a family CBM32 from Clostridium perfringens NagH binds N-acetyl-glucosamine in the primary subsite but can accommodate N-acetyl-galactosamine or mannose in the secondary site [31]. There are several examples of ligand promiscuity within family CBM32. In plant cell wall recognizing CBMs, they are often able to accommodate both cellulose and hemicelluloses. For example, several family CBM6 members interact with cellulose, xylose or laminarin [15, 32]. A family CBM41 can accommodate both alpha-1,4- and alpha-1,6-linked glucose found in amylopectin (from starch/glycogen) [33]. The flexibility in carbohydrate recognition by CBMs contributes to the targeting efficiency of carbohydrate-active enzymes in environments where there is diverse range of polysaccharides present (such as the plant cell wall or mammalian tissues).
CBMs and Multivalency
CBMs and Lectins
Several lectins [34, 35] fall into the CBM classification system in the Carbohydrate Active enZyme database as they exhibit similar folds and carbohydrate binding properties with CBMs. For example, ricin toxin B chain from Ricinus communis resides in family CBM13, while wheat germ agglutinin (WGA) can be found in family CBM18. The human lectin malectin is classified as family CBM57 and plays a role in N-linked glycan processing of polypeptides in the endoplasmic reticulum [36]. CBMs also share properties with lectins that are not yet incorporated in the Carbohydrate Active enZyme database. For example the fucose-specific Anquila anguila lectin AAA is similar to Type C CBMs found in family CBM6 and CBM32 [15]. Lectins which are classified as CBMs are incorporated into a family because they were found to share amino acid sequence identity with a known CBM appended to a carbohydrate-active enzyme (see criteria).
The most prominent feature that separates CBMs from lectins is the involvement of lectins in agglutination of sugar-containing molecules or glycoconjugates. Lectins often form quaternary structures as homodimers, trimers or tetramers with several binding sites which then agglutinate the target glycocongugate [34, 35]. CBMs themselves are not involved in the formation of quaternary structures and do not have agglutinating properties.
Lectins are also thought to have a higher density of hydrogen bond formation with their carbohydrate ligand than CBMs and may differ in their ability to utilize thermodynamic contributions from solvent rearrangement during ligand binding (references).
An excellent, brief historical overview of the discovery and characterization of lectins is available [34].
Criteria for Defining a new CBM family
In order to define a new CBM family, one must:
- Demonstrate an independent module as part of a larger carbohydrate-active enzyme.
- Demonstrate binding to carbohydrate ligand.
- Additional family members are then determined based on amino acid sequence similarity. To be defined as a true CBM, it must form part of a larger amino acid sequence encoding a putative CAZyme (or enzyme with demonstrated activity on a carbohydrate-containing substrate and the CBM enhances the catalytic efficiency of the enzyme by binding with or in close proximity of the substrate).
Amino acid sequence-based classification of a CBM family may lead to the incorporation of other carbohydrate binding proteins within a given family, including lectins (such as ricin (CBM13), tachycitin (CBM14), wheat germ agglutinin (CBM18), fucolectin (CBM47), and malectin (CBM57)) and periplasmic solute binding proteins (such as CBM32). The community is open to incorporation of all carbohydrate-binding proteins within the CBM classification system based on the above criteria.
Studying CBM-ligand Interactions
A great review on laboratory approaches to studying the binding function of carbohydrate-binding modules is available [37]. Typically, molecular biology techniques are used to overproduce a CBM protein in a host strain such as Escherichia coli which is then isolated and purified. Initial screening for carbohydrate binding interactions can be performed using screening techniques such as microarrays [25] or fluorescence microscopy techniques [21, 25, 38]. Several approaches can be taken to verify and quantify CBM-polysaccharide interaction, including affinity gel electrophoresis, UV difference and fluorescence spectroscopy, solid state depletion assay and isothermal titration calorimetry [39].
Overall demonstration of carbohydrate binding function by CBMs is essential to understanding how these associated modules confer enzymatic specificity to carbohydrate-active enzymes.
Biotechnological applications of CBMs
CBMs and their carbohydrate-binding properties are used for many different biological applications. Below is listed several examples.
- Features of CBMs are currently being exploited to create designer CAZymes with enhanced or modified carbohydrate recognition functions [40, 41, 42, 43].
- Family CBM9 can be used as an affinity tag to purify tagged proteins on a cellulose-based affinity column [44].
- CBMs are used as molecular probes to detect presence of specific carbohydrate motifs in plant [21, 38] and mammalian tissues [33, 45].
- CBMs are used in fibre modification. Engineered CBMs have been shown to increase the strength of cellulose pulp in paper-making processes [46, 47], in crosslinking polysaccharide fibres for biomaterials [48] and cotton fibre modification [49].
- There are several examples of CBMs being used to immobilize whole cells onto carbohydrate surfaces [50, 51, 52].
- CBMs are used to enhance bioprocessing enzymes for industrial uses in pulp processing and biofuel production [23, 53, 54].
- Starch binding CBMs added onto transglucosylating enzyme CGTase from GH13 created a fusion enzyme with more efficient transglucosylating activity with soluble starch, important for industrial biotransformation processes [55].
References
-
Davies, G.J. and Sinnott, M.L. (2008) Sorting the diverse: the sequence-based classifications of carbohydrate-active enzymes. Biochem. J. (BJ Classic Paper, online only). DOI: 10.1042/BJ20080382
- Cantarel BL, Coutinho PM, Rancurel C, Bernard T, Lombard V, and Henrissat B. (2009). The Carbohydrate-Active EnZymes database (CAZy): an expert resource for Glycogenomics. Nucleic Acids Res. 2009;37(Database issue):D233-8. DOI:10.1093/nar/gkn663 |
- Boraston AB, Bolam DN, Gilbert HJ, and Davies GJ. (2004). Carbohydrate-binding modules: fine-tuning polysaccharide recognition. Biochem J. 2004;382(Pt 3):769-81. DOI:10.1042/BJ20040892 |
- Hashimoto H (2006). Recent structural studies of carbohydrate-binding modules. Cell Mol Life Sci. 2006;63(24):2954-67. DOI:10.1007/s00018-006-6195-3 |
- Shoseyov O, Shani Z, and Levy I. (2006). Carbohydrate binding modules: biochemical properties and novel applications. Microbiol Mol Biol Rev. 2006;70(2):283-95. DOI:10.1128/MMBR.00028-05 |
- Guillén D, Sánchez S, and Rodríguez-Sanoja R. (2010). Carbohydrate-binding domains: multiplicity of biological roles. Appl Microbiol Biotechnol. 2010;85(5):1241-9. DOI:10.1007/s00253-009-2331-y |
- Gaskell A, Crennell S, and Taylor G. (1995). The three domains of a bacterial sialidase: a beta-propeller, an immunoglobulin module and a galactose-binding jelly-roll. Structure. 1995;3(11):1197-205. DOI:10.1016/s0969-2126(01)00255-6 |
- Georgelis N, Tabuchi A, Nikolaidis N, and Cosgrove DJ. (2011). Structure-function analysis of the bacterial expansin EXLX1. J Biol Chem. 2011;286(19):16814-23. DOI:10.1074/jbc.M111.225037 |
- Ficko-Blean E, Gregg KJ, Adams JJ, Hehemann JH, Czjzek M, Smith SP, and Boraston AB. (2009). Portrait of an enzyme, a complete structural analysis of a multimodular {beta}-N-acetylglucosaminidase from Clostridium perfringens. J Biol Chem. 2009;284(15):9876-84. DOI:10.1074/jbc.M808954200 |
-
Van Tilbeurgh, H., Tomme P., Claeyssens M., Bhikhabhai R., Pettersson G.(1986) Limited proteolysis of the cellobiohydrolase I from Trichoderma reesei. FEBS Lett. 204,223–227. [1]
- Tomme P, Van Tilbeurgh H, Pettersson G, Van Damme J, Vandekerckhove J, Knowles J, Teeri T, and Claeyssens M. (1988). Studies of the cellulolytic system of Trichoderma reesei QM 9414. Analysis of domain function in two cellobiohydrolases by limited proteolysis. Eur J Biochem. 1988;170(3):575-81. DOI:10.1111/j.1432-1033.1988.tb13736.x |
- Gilkes NR, Warren RA, Miller RC Jr, and Kilburn DG. (1988). Precise excision of the cellulose binding domains from two Cellulomonas fimi cellulases by a homologous protease and the effect on catalysis. J Biol Chem. 1988;263(21):10401-7. | Google Books | Open Library
-
Tomme, P., Warren, R.A., Miller, R.C., Jr., Kilburn, D.G. & Gilkes, N.R. (1995) in Enzymatic Degradation of Insoluble Polysaccharides (Saddler, J.N. & Penner, M., eds.), Cellulose-binding domains: classification and properties. pp. 142-163, American Chemical Society, Washington.
- Boraston AB, Revett TJ, Boraston CM, Nurizzo D, and Davies GJ. (2003). Structural and thermodynamic dissection of specific mannan recognition by a carbohydrate binding module, TmCBM27. Structure. 2003;11(6):665-75. DOI:10.1016/s0969-2126(03)00100-x |
- Boraston AB, Notenboom V, Warren RA, Kilburn DG, Rose DR, and Davies G. (2003). Structure and ligand binding of carbohydrate-binding module CsCBM6-3 reveals similarities with fucose-specific lectins and "galactose-binding" domains. J Mol Biol. 2003;327(3):659-69. DOI:10.1016/s0022-2836(03)00152-9 |
- Pires VM, Henshaw JL, Prates JA, Bolam DN, Ferreira LM, Fontes CM, Henrissat B, Planas A, Gilbert HJ, and Czjzek M. (2004). The crystal structure of the family 6 carbohydrate binding module from Cellvibrio mixtus endoglucanase 5a in complex with oligosaccharides reveals two distinct binding sites with different ligand specificities. J Biol Chem. 2004;279(20):21560-8. DOI:10.1074/jbc.M401599200 |
- Lawson CL, van Montfort R, Strokopytov B, Rozeboom HJ, Kalk KH, de Vries GE, Penninga D, Dijkhuizen L, and Dijkstra BW. (1994). Nucleotide sequence and X-ray structure of cyclodextrin glycosyltransferase from Bacillus circulans strain 251 in a maltose-dependent crystal form. J Mol Biol. 1994;236(2):590-600. DOI:10.1006/jmbi.1994.1168 |
- Fujimoto Z (2013). Structure and function of carbohydrate-binding module families 13 and 42 of glycoside hydrolases, comprising a β-trefoil fold. Biosci Biotechnol Biochem. 2013;77(7):1363-71. DOI:10.1271/bbb.130183 |
- Nakamura T, Mine S, Hagihara Y, Ishikawa K, Ikegami T, and Uegaki K. (2008). Tertiary structure and carbohydrate recognition by the chitin-binding domain of a hyperthermophilic chitinase from Pyrococcus furiosus. J Mol Biol. 2008;381(3):670-80. DOI:10.1016/j.jmb.2008.06.006 |
- Gilbert HJ, Knox JP, and Boraston AB. (2013). Advances in understanding the molecular basis of plant cell wall polysaccharide recognition by carbohydrate-binding modules. Curr Opin Struct Biol. 2013;23(5):669-77. DOI:10.1016/j.sbi.2013.05.005 |
- Hervé C, Rogowski A, Blake AW, Marcus SE, Gilbert HJ, and Knox JP. (2010). Carbohydrate-binding modules promote the enzymatic deconstruction of intact plant cell walls by targeting and proximity effects. Proc Natl Acad Sci U S A. 2010;107(34):15293-8. DOI:10.1073/pnas.1005732107 |
-
Din, N., Gilkes, N.R., Tekant, B., Miller, R.C., Jr., Warren, R.A., and Kilburn, D.G. (1991) Non-Hydrolytic Disruption of Cellulose Fibres by the Binding Domain of a Bacterial Cellulase. Nat. Biotech. 9, 1096 - 1099. http://doi:10.1038/nbt1191-1096
- Gourlay K, Arantes V, and Saddler JN. (2012). Use of substructure-specific carbohydrate binding modules to track changes in cellulose accessibility and surface morphology during the amorphogenesis step of enzymatic hydrolysis. Biotechnol Biofuels. 2012;5(1):51. DOI:10.1186/1754-6834-5-51 |
- Southall SM, Simpson PJ, Gilbert HJ, Williamson G, and Williamson MP. (1999). The starch-binding domain from glucoamylase disrupts the structure of starch. FEBS Lett. 1999;447(1):58-60. DOI:10.1016/s0014-5793(99)00263-x |
- van Bueren AL, Higgins M, Wang D, Burke RD, and Boraston AB. (2007). Identification and structural basis of binding to host lung glycogen by streptococcal virulence factors. Nat Struct Mol Biol. 2007;14(1):76-84. DOI:10.1038/nsmb1187 |
- Vaaje-Kolstad G, Westereng B, Horn SJ, Liu Z, Zhai H, Sørlie M, and Eijsink VG. (2010). An oxidative enzyme boosting the enzymatic conversion of recalcitrant polysaccharides. Science. 2010;330(6001):219-22. DOI:10.1126/science.1192231 |
- Montanier C, van Bueren AL, Dumon C, Flint JE, Correia MA, Prates JA, Firbank SJ, Lewis RJ, Grondin GG, Ghinet MG, Gloster TM, Herve C, Knox JP, Talbot BG, Turkenburg JP, Kerovuo J, Brzezinski R, Fontes CM, Davies GJ, Boraston AB, and Gilbert HJ. (2009). Evidence that family 35 carbohydrate binding modules display conserved specificity but divergent function. Proc Natl Acad Sci U S A. 2009;106(9):3065-70. DOI:10.1073/pnas.0808972106 |
- Meyer EA, Castellano RK, and Diederich F. (2003). Interactions with aromatic rings in chemical and biological recognition. Angew Chem Int Ed Engl. 2003;42(11):1210-50. DOI:10.1002/anie.200390319 |
- Jamal-Talabani S, Boraston AB, Turkenburg JP, Tarbouriech N, Ducros VM, and Davies GJ. (2004). Ab initio structure determination and functional characterization of CBM36; a new family of calcium-dependent carbohydrate binding modules. Structure. 2004;12(7):1177-87. DOI:10.1016/j.str.2004.04.022 |
- Montanier C, Flint JE, Bolam DN, Xie H, Liu Z, Rogowski A, Weiner DP, Ratnaparkhe S, Nurizzo D, Roberts SM, Turkenburg JP, Davies GJ, and Gilbert HJ. (2010). Circular permutation provides an evolutionary link between two families of calcium-dependent carbohydrate binding modules. J Biol Chem. 2010;285(41):31742-54. DOI:10.1074/jbc.M110.142133 |
- Ficko-Blean E and Boraston AB. (2009). N-acetylglucosamine recognition by a family 32 carbohydrate-binding module from Clostridium perfringens NagH. J Mol Biol. 2009;390(2):208-20. DOI:10.1016/j.jmb.2009.04.066 |
- van Bueren AL, Morland C, Gilbert HJ, and Boraston AB. (2005). Family 6 carbohydrate binding modules recognize the non-reducing end of beta-1,3-linked glucans by presenting a unique ligand binding surface. J Biol Chem. 2005;280(1):530-7. DOI:10.1074/jbc.M410113200 |
- van Bueren AL and Boraston AB. (2007). The structural basis of alpha-glucan recognition by a family 41 carbohydrate-binding module from Thermotoga maritima. J Mol Biol. 2007;365(3):555-60. DOI:10.1016/j.jmb.2006.10.018 |
- Sharon N and Lis H. (2004). History of lectins: from hemagglutinins to biological recognition molecules. Glycobiology. 2004;14(11):53R-62R. DOI:10.1093/glycob/cwh122 |
- Nathan Sharon and H. Lis. (2007-10) Lectins. Springer Science & Business Media.
- Galli C, Bernasconi R, Soldà T, Calanca V, and Molinari M. (2011). Malectin participates in a backup glycoprotein quality control pathway in the mammalian ER. PLoS One. 2011;6(1):e16304. DOI:10.1371/journal.pone.0016304 |
- Abbott DW and Boraston AB. (2012). Quantitative approaches to the analysis of carbohydrate-binding module function. Methods Enzymol. 2012;510:211-31. DOI:10.1016/B978-0-12-415931-0.00011-2 |
- McCartney L, Blake AW, Flint J, Bolam DN, Boraston AB, Gilbert HJ, and Knox JP. (2006). Differential recognition of plant cell walls by microbial xylan-specific carbohydrate-binding modules. Proc Natl Acad Sci U S A. 2006;103(12):4765-70. DOI:10.1073/pnas.0508887103 |
- Lammerts van Bueren A and Boraston AB. (2004). Binding sub-site dissection of a carbohydrate-binding module reveals the contribution of entropy to oligosaccharide recognition at "non-primary" binding subsites. J Mol Biol. 2004;340(4):869-79. DOI:10.1016/j.jmb.2004.05.038 |
- Cuskin F, Flint JE, Gloster TM, Morland C, Baslé A, Henrissat B, Coutinho PM, Strazzulli A, Solovyova AS, Davies GJ, and Gilbert HJ. (2012). How nature can exploit nonspecific catalytic and carbohydrate binding modules to create enzymatic specificity. Proc Natl Acad Sci U S A. 2012;109(51):20889-94. DOI:10.1073/pnas.1212034109 |
- McKee LS, Peña MJ, Rogowski A, Jackson A, Lewis RJ, York WS, Krogh KB, Viksø-Nielsen A, Skjøt M, Gilbert HJ, and Marles-Wright J. (2012). Introducing endo-xylanase activity into an exo-acting arabinofuranosidase that targets side chains. Proc Natl Acad Sci U S A. 2012;109(17):6537-42. DOI:10.1073/pnas.1117686109 |
- Tang CD, Li JF, Wei XH, Min R, Gao SJ, Wang JQ, Yin X, and Wu MC. (2013). Fusing a carbohydrate-binding module into the Aspergillus usamii β-mannanase to improve its thermostability and cellulose-binding capacity by in silico design. PLoS One. 2013;8(5):e64766. DOI:10.1371/journal.pone.0064766 |
- Kavoosi M, Meijer J, Kwan E, Creagh AL, Kilburn DG, and Haynes CA. (2004). Inexpensive one-step purification of polypeptides expressed in Escherichia coli as fusions with the family 9 carbohydrate-binding module of xylanase 10A from T. maritima. J Chromatogr B Analyt Technol Biomed Life Sci. 2004;807(1):87-94. DOI:10.1016/j.jchromb.2004.03.031 |
- Boraston AB, Wang D, and Burke RD. (2006). Blood group antigen recognition by a Streptococcus pneumoniae virulence factor. J Biol Chem. 2006;281(46):35263-71. DOI:10.1074/jbc.M607620200 |
-
Levy, I., Paldi, T., Siegel, D., and Shoseyov, O. (2003) Cellulose binding domain from Clostridium cellulovorans as a paper modification reagent. Nordic Pulp Paper Res. J. 18:421-428.
-
Yokota, S., Matuso, K., Kitaoka, T., and Wariishi, H. (2009) Retention and paper strength characteristics of anionic polyacrylamides conjugated with carbohydrate-binding modules. "Carbohydrate-binding anionic PAM". BioResources 4(1):234-244.
- Levy I, Paldi T, and Shoseyov O. (2004). Engineering a bifunctional starch-cellulose cross-bridge protein. Biomaterials. 2004;25(10):1841-9. DOI:10.1016/j.biomaterials.2003.08.041 |
-
Zhang, Y., Chen, S., He, M., Wu, J., Chen, J., and Wang, Q. (2011) Effects of Thermobifida fusca Cutinase-carbohydrate-binding Module Fusion Proteins on Cotton Bioscouring. Biotechnology and Bioprocess Engineering. 16,645-653 http://DOI:10.1007/s12257-011-0036-4
- Francisco JA, Stathopoulos C, Warren RA, Kilburn DG, and Georgiou G. (1993). Specific adhesion and hydrolysis of cellulose by intact Escherichia coli expressing surface anchored cellulase or cellulose binding domains. Biotechnology (N Y). 1993;11(4):491-5. DOI:10.1038/nbt0493-491 |
- Simşek Ö, Sabanoğlu S, Çon AH, Karasu N, Akçelik M, and Saris PE. (2013). Immobilization of nisin producer Lactococcus lactis strains to chitin with surface-displayed chitin-binding domain. Appl Microbiol Biotechnol. 2013;97(10):4577-87. DOI:10.1007/s00253-013-4700-9 |
- Wang JY and Chao YP. (2006). Immobilization of cells with surface-displayed chitin-binding domain. Appl Environ Microbiol. 2006;72(1):927-31. DOI:10.1128/AEM.72.1.927-931.2006 |
- Reyes-Ortiz V, Heins RA, Cheng G, Kim EY, Vernon BC, Elandt RB, Adams PD, Sale KL, Hadi MZ, Simmons BA, Kent MS, and Tullman-Ercek D. (2013). Addition of a carbohydrate-binding module enhances cellulase penetration into cellulose substrates. Biotechnol Biofuels. 2013;6(1):93. DOI:10.1186/1754-6834-6-93 |
- Ravalason H, Herpoël-Gimbert I, Record E, Bertaud F, Grisel S, de Weert S, van den Hondel CA, Asther M, Petit-Conil M, and Sigoillot JC. (2009). Fusion of a family 1 carbohydrate binding module of Aspergillus niger to the Pycnoporus cinnabarinus laccase for efficient softwood kraft pulp biobleaching. J Biotechnol. 2009;142(3-4):220-6. DOI:10.1016/j.jbiotec.2009.04.013 |
- Han R, Li J, Shin HD, Chen RR, Du G, Liu L, and Chen J. (2013). Carbohydrate-binding module-cyclodextrin glycosyltransferase fusion enables efficient synthesis of 2-O-d-glucopyranosyl-l-ascorbic acid with soluble starch as the glycosyl donor. Appl Environ Microbiol. 2013;79(10):3234-40. DOI:10.1128/AEM.00363-13 |