CAZypedia needs your help! We have many unassigned GH, PL, CE, AA, GT, and CBM pages in need of Authors and Responsible Curators.
Scientists at all career stages, including students, are welcome to contribute to CAZypedia. Read more here, and in the 10th anniversary article in Glycobiology.
New to the CAZy classification? Read this first.
*
Consider attending the 15th Carbohydrate Bioengineering Meeting in Ghent, 5-8 May 2024.
Difference between revisions of "Auxiliary Activity Family 3"
Line 33: | Line 33: | ||
Enzymes of the CAZY family AA3 are widespread and catalyse the oxidation of alcohols or carbohydrates with the concomitant formation of hydrogen peroxide or hydroquinones <cite>Levasseur2013</cite>. AA3 enzymes are most abundant in wood-degrading fungi where they typically display a high multigenicity <cite>Sützl2018</cite>. The main function of fungal AA3 enzymes is the stimulation of lignocellulose degradation in cooperation with other AA-enzymes such as peroxidases (AA2) <cite>Hernandez2012</cite> or lytic polysaccharide monooxygenases (AA9) <cite> Kracher2016, Garajova2016 </cite>. In yeast, AA3 enzymes are involved in the catabolism of alcohols <cite> Goswami2013</cite>, and some AA3 genes identified in insects are thought to be relevant for immunity and development <cite> Iida2007, Sun2012</cite>. Recently, a bacterial AA3 enzyme with unknown biological function was isolated and characterized <cite> Mendes2017 </cite>. The functionally diverse enzymes of family AA3 all belong to the structurally related glucose-methanol-choline (GMC) family of oxidoreductases and require a flavin-adenine dinucleotide (FAD) cofactor for catalytic activity. Based on their sequences members of the AA3 family were divided into four subfamilies in the CAZy database (Figure 1). Family AA3_1 contains the flavodehydrogenase domain of cellobiose dehydrogenase ([{{EClink}}1.1.99.18 EC 1.1.99.18]), family AA3_2 includes aryl alcohol oxidase ([{{EClink}}1.1.3.7 EC 1.1.3.7]), glucose oxidase ([{{EClink}}1.1.3.4 EC 1.1.3.4]), glucose dehydrogenase ([{{EClink}}1.1.5.9 EC 1.1.5.9]) and pyranose dehydrogenase ([{{EClink}}1.1.99.29 EC 1.1.99.29]), family AA3_3 consists of alcohol (methanol) oxidases ([{{EClink}}1.1.3.13 EC 1.1.3.13]) and family AA3_4 comprises pyranose oxidoreductases ([{{EClink}}1.1.3.10 EC 1.1.3.10]). | Enzymes of the CAZY family AA3 are widespread and catalyse the oxidation of alcohols or carbohydrates with the concomitant formation of hydrogen peroxide or hydroquinones <cite>Levasseur2013</cite>. AA3 enzymes are most abundant in wood-degrading fungi where they typically display a high multigenicity <cite>Sützl2018</cite>. The main function of fungal AA3 enzymes is the stimulation of lignocellulose degradation in cooperation with other AA-enzymes such as peroxidases (AA2) <cite>Hernandez2012</cite> or lytic polysaccharide monooxygenases (AA9) <cite> Kracher2016, Garajova2016 </cite>. In yeast, AA3 enzymes are involved in the catabolism of alcohols <cite> Goswami2013</cite>, and some AA3 genes identified in insects are thought to be relevant for immunity and development <cite> Iida2007, Sun2012</cite>. Recently, a bacterial AA3 enzyme with unknown biological function was isolated and characterized <cite> Mendes2017 </cite>. The functionally diverse enzymes of family AA3 all belong to the structurally related glucose-methanol-choline (GMC) family of oxidoreductases and require a flavin-adenine dinucleotide (FAD) cofactor for catalytic activity. Based on their sequences members of the AA3 family were divided into four subfamilies in the CAZy database (Figure 1). Family AA3_1 contains the flavodehydrogenase domain of cellobiose dehydrogenase ([{{EClink}}1.1.99.18 EC 1.1.99.18]), family AA3_2 includes aryl alcohol oxidase ([{{EClink}}1.1.3.7 EC 1.1.3.7]), glucose oxidase ([{{EClink}}1.1.3.4 EC 1.1.3.4]), glucose dehydrogenase ([{{EClink}}1.1.5.9 EC 1.1.5.9]) and pyranose dehydrogenase ([{{EClink}}1.1.99.29 EC 1.1.99.29]), family AA3_3 consists of alcohol (methanol) oxidases ([{{EClink}}1.1.3.13 EC 1.1.3.13]) and family AA3_4 comprises pyranose oxidoreductases ([{{EClink}}1.1.3.10 EC 1.1.3.10]). | ||
− | == Subfamily | + | == Subfamily AA3_1: cellobiose dehydrogenase == |
− | + | Cellobiose dehydrogenases (CDHs) are extracellular flavocytochromes that were first described in 1974 <cite>Westermark1974</cite>. CDHs are exclusively found in wood-degrading and phytopathogenic fungi belonging to the phyla Basidiomycota (Class-I CDHs) and Ascomycota (Class-II and -III CDHs) <cite>Kracher2016b</cite>. They oxidize a wide variety of lignocellulose-derived saccharides to their corresponding sugar lactones. CDHs show a high preference for soluble, β-(1,4)-interlinked saccharides and scarcely oxidize monosaccharides. | |
− | Cellobiose | + | A common feature of all CDHs is their complex bipartite structure, which comprises a C-terminal cytochrome-binding domain (CYT) and a larger, catalytic flavodehydrogenase (DH) domain encoded within a single polypeptide chain <cite>Hallberg2002</cite> (Figure 2A). Both domains are connected by a flexible linker which typically comprises 15 – 30 amino acids. An important in vivo function of CDH is the reduction and activation of family AA9 lytic polysaccharide monooxygenases via its heme b domain <cite>Phillips2011, Tan2015</cite> 14,15. Recently, the holoenzyme structures of Neurospora crassa CDH (pdb: 4qi7) and Myriococcum thermophilum CDH (pdb: 4qi5) were reported. Two structures of N. crassa CDH showed an “open-state” conformation in which DH and CYT were spatially separated, whereas a structure of ''M. thermophilum'' CDH showed a “closed-state” conformation in which the propionate arm of the cytochrome domain interacted with the catalytic centre in DH <cite> Tan2015</cite>. Analysis by small angle scattering also suggested a number of possible intermediate conformers that exist in solution <cite>Tan2015, Bodenheimer2018</cite>. While the closed-state allows interdomain electron transfer from DH to CYT, reduction of electron acceptors (e.g. AA9 enzymes) might occur in the open-state, in which the heme cofactor is fully accessible. |
− | |||
− | |||
− | |||
− | |||
− | |||
− | |||
− | |||
− | |||
− | |||
− | |||
− | |||
− | |||
− | |||
− | |||
− | |||
− | |||
− | |||
− | |||
− | |||
Line 107: | Line 88: | ||
#Iida2007 pmid=17498303 | #Iida2007 pmid=17498303 | ||
#Sun2012 pmid=23022604 | #Sun2012 pmid=23022604 | ||
− | #Mendes2017 Mendes S, Banha C, Madeira J, Santos D, Miranda V, Manzanera M, Ventura MR, van Berkel WJH, Martins LO. Characterization of a bacterial pyranose 2-oxidase from Arthrobacter siccitolerans. 2016 J Mol Catal B Enzym 133, S34–S43 | + | #Mendes2017 Mendes S, Banha C, Madeira J, Santos D, Miranda V, Manzanera M, Ventura MR, van Berkel WJH, Martins LO. Characterization of a bacterial pyranose 2-oxidase from Arthrobacter siccitolerans. 2016 J Mol Catal B Enzym 133, S34–S43 |
+ | # Westermark1974 Westermark U, Eriksson KE. Cellobiose:Quinone Oxidoreductase, a New Wood-degrading Enzyme from White-rot Fungi. 1974 Acta Chem Scand 1974 28b:209–214. | ||
+ | #Kracher2016b Kracher D, Ludwig R. Cellobiose dehydrogenase: An essential enzyme for lignocellulose degradation in nature - A review. 2016 Bodenkultur 67:145–163. | ||
+ | #Hallberg2002 pmid=12493734 | ||
+ | #Phillips2011 pmid=22004347 | ||
+ | #Tan2015 pmid=26151670 | ||
+ | #Bodenheimer2018 pmid=29374564 | ||
</cite></biblio> | </cite></biblio> |
Revision as of 04:13, 2 May 2018
This page is currently under construction. This means that the Responsible Curator has deemed that the page's content is not quite up to CAZypedia's standards for full public consumption. All information should be considered to be under revision and may be subject to major changes.
- Author: ^^^Roland Ludwig^^^ and ^^^Daniel Kracher^^^
- Responsible Curator: ^^^Roland Ludwig^^^
Auxiliary Activity Family AA3 | |
Clan | AA3 |
Mechanism | FAD-dependent substrate oxidation |
Active site residues | known |
CAZy DB link | |
http://www.cazy.org/AA3.html |
General properties and substrate specificities
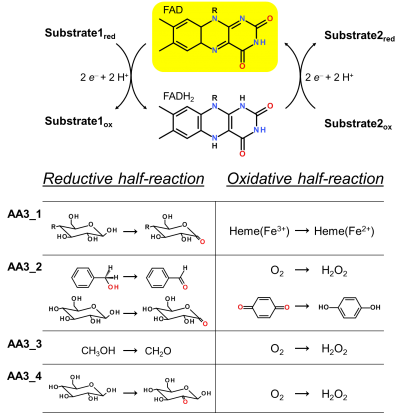
Enzymes of the CAZY family AA3 are widespread and catalyse the oxidation of alcohols or carbohydrates with the concomitant formation of hydrogen peroxide or hydroquinones [1]. AA3 enzymes are most abundant in wood-degrading fungi where they typically display a high multigenicity [2, 3]. The main function of fungal AA3 enzymes is the stimulation of lignocellulose degradation in cooperation with other AA-enzymes such as peroxidases (AA2) [4] or lytic polysaccharide monooxygenases (AA9) [5, 6]. In yeast, AA3 enzymes are involved in the catabolism of alcohols [7], and some AA3 genes identified in insects are thought to be relevant for immunity and development [8, 9]. Recently, a bacterial AA3 enzyme with unknown biological function was isolated and characterized [10]. The functionally diverse enzymes of family AA3 all belong to the structurally related glucose-methanol-choline (GMC) family of oxidoreductases and require a flavin-adenine dinucleotide (FAD) cofactor for catalytic activity. Based on their sequences members of the AA3 family were divided into four subfamilies in the CAZy database (Figure 1). Family AA3_1 contains the flavodehydrogenase domain of cellobiose dehydrogenase (EC 1.1.99.18), family AA3_2 includes aryl alcohol oxidase (EC 1.1.3.7), glucose oxidase (EC 1.1.3.4), glucose dehydrogenase (EC 1.1.5.9) and pyranose dehydrogenase (EC 1.1.99.29), family AA3_3 consists of alcohol (methanol) oxidases (EC 1.1.3.13) and family AA3_4 comprises pyranose oxidoreductases (EC 1.1.3.10).
Subfamily AA3_1: cellobiose dehydrogenase
Cellobiose dehydrogenases (CDHs) are extracellular flavocytochromes that were first described in 1974 [11]. CDHs are exclusively found in wood-degrading and phytopathogenic fungi belonging to the phyla Basidiomycota (Class-I CDHs) and Ascomycota (Class-II and -III CDHs) [12]. They oxidize a wide variety of lignocellulose-derived saccharides to their corresponding sugar lactones. CDHs show a high preference for soluble, β-(1,4)-interlinked saccharides and scarcely oxidize monosaccharides. A common feature of all CDHs is their complex bipartite structure, which comprises a C-terminal cytochrome-binding domain (CYT) and a larger, catalytic flavodehydrogenase (DH) domain encoded within a single polypeptide chain [13] (Figure 2A). Both domains are connected by a flexible linker which typically comprises 15 – 30 amino acids. An important in vivo function of CDH is the reduction and activation of family AA9 lytic polysaccharide monooxygenases via its heme b domain [14, 15] 14,15. Recently, the holoenzyme structures of Neurospora crassa CDH (pdb: 4qi7) and Myriococcum thermophilum CDH (pdb: 4qi5) were reported. Two structures of N. crassa CDH showed an “open-state” conformation in which DH and CYT were spatially separated, whereas a structure of M. thermophilum CDH showed a “closed-state” conformation in which the propionate arm of the cytochrome domain interacted with the catalytic centre in DH [15]. Analysis by small angle scattering also suggested a number of possible intermediate conformers that exist in solution [15, 16]. While the closed-state allows interdomain electron transfer from DH to CYT, reduction of electron acceptors (e.g. AA9 enzymes) might occur in the open-state, in which the heme cofactor is fully accessible.
Subfamily 2 - P2O
Kinetics and Mechanism
Pyranose 2-oxidase (P2O; syn. pyranose oxidase, POX; EC 1.1.3.10; pyranose:oxygen 2-oxidoreductase) is widespread in lignin-degrading white-rot fungi and catalyzes the oxygen dependent oxidation of several monosaccharides at the C2 position to the corresponding 2-keto-aldoses and H2O2 [17]. The preferred substrate is D-glucose, which is oxidized to 2-keto-D-glucose. Unlike other GMC-oxidoreductases, the FAD in P2O is covalently linked via its isoalloxazine ring 8α-methyl group to the N12 atom of His167 (histidyl linkage, [18]). The supposed physiological role of P2O is to generate H2O2 as a substrate for lignin-degrading peroxidases. P2O is localized in the hyphal periplasmatic space [19].
During the reductive half reaction, two electrons are transferred from the substrate to the FAD, leading to the formation of FADH2 and the 2-keto sugar. During the oxidative half reaction, the two stored electrons are transferred to molecular oxygen to form H2O2. The catalytic reaction is proposed to be of the ping-pong bi-bi type, since the 2-keto-sugar product is released prior to the reaction with oxygen [17]. The active site contains a conserved, catalytic His–Asn pair positioned below the FAD isoalloxazine ring, which is typical for GMC oxidoreductases. His XXX (PDB) or His YYY (PDB) is the catalytic base that facilitates deprotonation of the sugar substrate.
Catalytic Residues
The FAD cofactor in the flavodehydrogenase domain is covalently bound. The carbohydrate C2 atom is oriented towards N5 of the FAD isoalloxazine ring at a distance of approx. XY9 Å (PDB, Ref.3). Indispensible for catalysis is the general base histidine (HXXX in PDB, HYYY in PDB). A strictly conserved arginine residue (Argxxx in PDB, Arg YYY in PDB) is close to the caltalytic histidine [17].
Three-dimensional structures
Crystal structures of P2O from Trametes multicolor and Phanerochaete chrysosporium were resolved (pdb: 1TT0, [20];pdb: 4MIG; [21]) P2O is a homotetrameric enzyme with a molecular masses of approx. 68,000 per subunit. Each subunit contains the flavin-binding Rossmann-fold typical for GMC- oxidoreductases. The substrate-binding subdomain comprises six-stranded central-β sheet and three α-helices. The homotetramer features a large internal cavity, from which the four active sites are accessible. P2O is a non-glycosylated enzyme.
Family Firsts
- First P2O identified
- Pyranose oxidase was first isolated from the basidiomycete Polyporus obtusus in 1968 [22].
- First 3-D structure
- P2O from Trametes multicolor MB 49 (pdb: 1TT0; [20])
Subfamily 3 - GOx
Kinetics and Mechanism
Glucose oxidase (GOx, syn. GOD, EC 1.1.x.x., glucose:oxygen 1-oxidoreductase) catalyzes the regioselective oxidation of β-D-glucose to gluconic acid by utilizing molecular oxygen as an electron acceptor with simultaneous production of hydrogen peroxide. Glucose oxidases are produced by a number of fungi and insects. Proposed functions are related to the peroxide-producing abilities of the enzyme and include the preservation of honey, microbial defense as well as the support of H2O2 dependent ligninases in wood degrading fungi [23].
In the oxidative half reaction of GOx, two-electron oxidation of D-glucose at C1 results in the formation of gluconolactone and reduced cofactor (FADH2). Reduction by glucose occurs via a hydride ion transfer to the N-5 position of the FAD. Oxygen reduction to H2O2 involves the uptake of two electrons (from FADH2) and two protons. A highly conserved His (His516 in Aspergillus niger GOx) in the active site of the enzyme was identified by site directed mutagenesis as the catalytic base [24].
Catalytic Residues
Content is to be added here.
Three-dimensional structures
Content is to be added here.
Family Firsts
- First GOx identified
- Glucose oxidase from Aspergillus niger in 1928 [25].
- First 3D structure
- Glucose oxidase from Aspergillus niger (1GAL; [26]).
References
- Levasseur A, Drula E, Lombard V, Coutinho PM, and Henrissat B. (2013). Expansion of the enzymatic repertoire of the CAZy database to integrate auxiliary redox enzymes. Biotechnol Biofuels. 2013;6(1):41. DOI:10.1186/1754-6834-6-41 |
-
ützl2018 pmid=29411063
-
pmid= 22249717
- Kracher D, Scheiblbrandner S, Felice AK, Breslmayr E, Preims M, Ludwicka K, Haltrich D, Eijsink VG, and Ludwig R. (2016). Extracellular electron transfer systems fuel cellulose oxidative degradation. Science. 2016;352(6289):1098-101. DOI:10.1126/science.aaf3165 |
-
pmid= 27312718
- Goswami P, Chinnadayyala SS, Chakraborty M, Kumar AK, and Kakoti A. (2013). An overview on alcohol oxidases and their potential applications. Appl Microbiol Biotechnol. 2013;97(10):4259-75. DOI:10.1007/s00253-013-4842-9 |
- Iida K, Cox-Foster DL, Yang X, Ko WY, and Cavener DR. (2007). Expansion and evolution of insect GMC oxidoreductases. BMC Evol Biol. 2007;7:75. DOI:10.1186/1471-2148-7-75 |
- Sun W, Shen YH, Yang WJ, Cao YF, Xiang ZH, and Zhang Z. (2012). Expansion of the silkworm GMC oxidoreductase genes is associated with immunity. Insect Biochem Mol Biol. 2012;42(12):935-45. DOI:10.1016/j.ibmb.2012.09.006 |
-
Mendes S, Banha C, Madeira J, Santos D, Miranda V, Manzanera M, Ventura MR, van Berkel WJH, Martins LO. Characterization of a bacterial pyranose 2-oxidase from Arthrobacter siccitolerans. 2016 J Mol Catal B Enzym 133, S34–S43
-
Westermark U, Eriksson KE. Cellobiose:Quinone Oxidoreductase, a New Wood-degrading Enzyme from White-rot Fungi. 1974 Acta Chem Scand 1974 28b:209–214.
-
Kracher D, Ludwig R. Cellobiose dehydrogenase: An essential enzyme for lignocellulose degradation in nature - A review. 2016 Bodenkultur 67:145–163.
- Hallberg BM, Henriksson G, Pettersson G, Vasella A, and Divne C. (2003). Mechanism of the reductive half-reaction in cellobiose dehydrogenase. J Biol Chem. 2003;278(9):7160-6. DOI:10.1074/jbc.M210961200 |
- Phillips CM, Beeson WT, Cate JH, and Marletta MA. (2011). Cellobiose dehydrogenase and a copper-dependent polysaccharide monooxygenase potentiate cellulose degradation by Neurospora crassa. ACS Chem Biol. 2011;6(12):1399-406. DOI:10.1021/cb200351y |
- Tan TC, Kracher D, Gandini R, Sygmund C, Kittl R, Haltrich D, Hällberg BM, Ludwig R, and Divne C. (2015). Structural basis for cellobiose dehydrogenase action during oxidative cellulose degradation. Nat Commun. 2015;6:7542. DOI:10.1038/ncomms8542 |
- Bodenheimer AM, O'Dell WB, Oliver RC, Qian S, Stanley CB, and Meilleur F. (2018). Structural investigation of cellobiose dehydrogenase IIA: Insights from small angle scattering into intra- and intermolecular electron transfer mechanisms. Biochim Biophys Acta Gen Subj. 2018;1862(4):1031-1039. DOI:10.1016/j.bbagen.2018.01.016 |